What is the epithelial to mesenchymal transition?
Epithelial to mesenchymal transition (EMT) is the process whereby epithelial cells are transformed into mesenchymal cells. Epithelial cells form the epithelium tissue which covers the internal and external body surface of an organism. These cells are polarized and form extensive cell-cell adhesions, including adherens junctions and tight junctions, with each other [1][2]. One of the fundamental functions of epithelial tissue is to act as a protective barrier for the underlying tissue and organs. Mesenchymal cells are, however, not well organized in a three-dimensional extracellular matrix [3]. During development, mesenchymal cells can gain the ability to migrate and differentiate into other cell types. This gives rise to the formation of defined structures, such as the neural crest [3].
During EMT, epithelial cells lose their polarity, as well as their cell-cell adhesions, and gain the ability to migrate, proliferate, differentiate and develop into specific tissues and organs. EMT is classified into 3 main types:
- Type 1 EMT is associated with development such as embryo formation, and organ development;
- Type 2 EMT is associated with repair processes such as wound healing, tissue regeneration, and organ fibrosis;
- Type 3 EMT is associated with tumor metastasis [3][4].
Here we will only consider the EMT in development where it plays important roles in producing the various tissues and organs, and defining their arrangement in the body. Importantly, EMT is reversible, meaning a mesenchymal to epithelial transition, (MET) can occur. In fact, several cycles of EMT and MET are required before the final architecture of the organ is established. These rounds of EMT are known as primary, secondary and tertiary EMT [3]. An example of primary EMT occurs during gastrulation, where embryonic epithelium undergoes EMT to form the mesoderm. In vertebrates, gastrulation is induced by proteins from the transforming growth factor β (TGFβ) superfamily, especially Nodal and Vg1 [5][6]. These TGFβ proteins, together with the aid of fibroblast growth factor (FGFs) signaling, induce the Snail gene [7][8][9], which is a key transcription factor for EMT [10]. Snail promotes the breaking away of the epithelial cells from the basal membrane prior to their EMT, by repressing E-cadherin expression [11] and activating metalloproteinases [8]. This cleaves the cell adhesion complexes and degrades extracellular matrix, respectively, to aid cell migration [10]. Nodal and Vg1 also promotes Wnt signalling [6][12], which co-operates with FGF and Snail to orchestrate EMT [9].
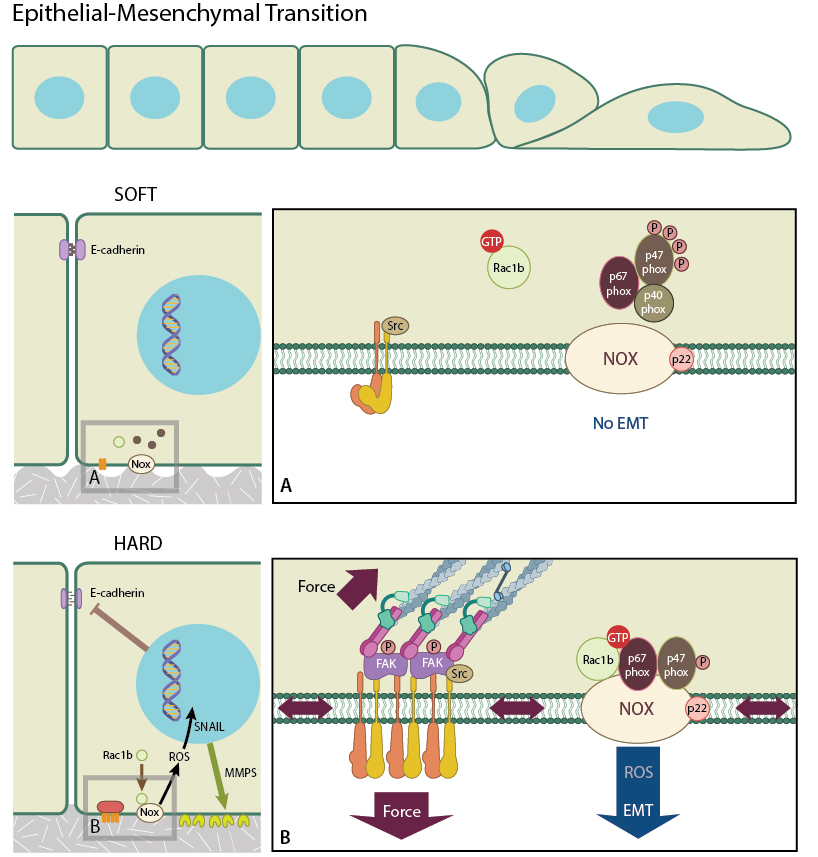
Stiff matrix promotes integrin clustering which promotes the recruitment of Rac1b to the membrane, facilitating its interaction with NADPH oxidase and the subsequent production of ROCs which promote Snail expression and hence EMT. On the other hand, Rac1b is less likely to be recruited to the membrane of cells in a softer matrix. EMT is less likely to occur in this situation.
Physical properties regulate EMT
The molecular mechanisms that drive EMT are complex and involve many more biochemical components. These have been reviewed extensively in [10]. In addition to the biochemical signals, mechanical signals, such as the rigidity of the cell’s microenvironment, also play roles in the regulation of EMT. Lee et al. observed that mammary epithelial cells underwent EMT when placed on hard substrates and treated with matrix metalloproteinase-3 (MMP3). This was not observed in cells grown on soft substrates [13]. Furthermore, treatment with MMP3, caused cells on both substrates to increase their expression of Rac1b. However, only those cells on the hard substrate produced reactive oxygen species (ROS) [13] which is downstream of MMP3 and Rac1b [14]. Interestingly, in cells on hard substrates, Rac1b is recruited to the cell membrane through integrin clustering. This allows Rac1b to assemble with NADPH oxidase and contribute to ROS production. It was determined therefore, that cells growing on rigid substrates will undergo EMT after the expression of Snail is increased following Rac1b mediated ROS stimulation [14]. Stiffer substrates have been shown to promote integrin clustering [14] and focal adhesion formation [15], and it is this process that leads to Rac1b recruitment and its interaction with NADPH oxidase that produces ROS, which in turn promotes EMT. As soft substrates do not promote integrin clustering, this cascade of events does not occur, and cells growing on soft substrates do not undergo EMT.
Besides the rigidity of the cell microenvironment, other mechanical stresses placed on epithelial cells from their neighbouring cells, are implicated in the initiation of EMT. This was described by Gomez et al. who used microfabrication techniques to generate a confluent, square-shaped sheet of mammary epithelial cells. After treating them with TGFβ (an inducer of EMT), the team observed that the cells underwent EMT preferentially at the corner and edge of the square, which correspond to regions of higher mechanical stress [16]. High levels of myocardin-related transcription factors A (MRTFA) was also found to be localized in the nucleus of cells located at regions of higher mechanical strain [16].
When mechanical strain is lower, MRTFA binds to actin monomers and is retained in the cytoplasm [17]. However, when mechanical stress increases, the tension of cytoskeleton increases and this activates the Rho-actin signaling pathway, which reduces the amount of monomeric actin in the cytoplasm, causing MRTFA to translocate to the nucleus [17][18]. Here, MRTFA forms a complex with Smad3, which binds to the promoter region of the SNAI2 gene, activating its transcription and subsequently dissolving cell-cell contacts [19]. MRTFA is a co-activator of serum response factor (SRF) which upregulates actin cytoskeletal proteins leading to cytoskeleton remodelling [19]. This dual pathway is activated following the nuclear localization of MRTFA, and promotes epithelial cells to undergo EMT.
The direct application of biomechanical force has also been found to be sufficient to induce EMT. Zhou et al. stretched human keratinocytes by applying a 10% elongation to these cells, and observed that the applied force can directly activate NF-κB, which in turn promotes EMT [20][21][22]. Similarly, the application of compressive force to HEK-293 cells was shown to induce the expression of EMT regulators SNAI2 and ZEB1, along with the messenchymal marker VIM, via the RhoA/ROCK pathway [23]. Taken together, these experimental results highlight that underlying EMT is a complex network of signalling that involves not only biochemical signals, but also a variety of mechanical cues.
References
- Gumbiner BM. Cell adhesion: the molecular basis of tissue architecture and morphogenesis. Cell 1996; 84(3):345-57. [PMID: 8608588]
- Niessen CM. Tight junctions/adherens junctions: basic structure and function. J. Invest. Dermatol. 2007; 127(11):2525-32. [PMID: 17934504]
- Thiery JP, Acloque H, Huang RYJ, and Nieto MA. Epithelial-mesenchymal transitions in development and disease. Cell 2009; 139(5):871-90. [PMID: 19945376]
- Kalluri R, and Weinberg RA. The basics of epithelial-mesenchymal transition. J. Clin. Invest. 2009; 119(6):1420-8. [PMID: 19487818]
- Chea HK, Wright CV, and Swalla BJ. Nodal signaling and the evolution of deuterostome gastrulation. Dev. Dyn. 2005; 234(2):269-78. [PMID: 16127715]
- Skromne I, and Stern CD. A hierarchy of gene expression accompanying induction of the primitive streak by Vg1 in the chick embryo. Mech. Dev. 2002; 114(1-2):115-8. [PMID: 12175495]
- van Meeteren LA, and ten Dijke P. Regulation of endothelial cell plasticity by TGF-β. Cell Tissue Res. 2011; 347(1):177-86. [PMID: 21866313]
- Barrallo-Gimeno A, and Nieto MA. The Snail genes as inducers of cell movement and survival: implications in development and cancer. Development 2005; 132(14):3151-61. [PMID: 15983400]
- Ciruna B, and Rossant J. FGF signaling regulates mesoderm cell fate specification and morphogenetic movement at the primitive streak. Dev. Cell 2001; 1(1):37-49. [PMID: 11703922]
- Lamouille S, Xu J, and Derynck R. Molecular mechanisms of epithelial-mesenchymal transition. Nat Rev Mol Cell Biol 2014; 15(3):178-96. [PMID: 24556840]
- Cano A, Pérez-Moreno MA, Rodrigo I, Locascio A, Blanco MJ, del Barrio MG, Portillo F, and Nieto MA. The transcription factor snail controls epithelial-mesenchymal transitions by repressing E-cadherin expression. Nat. Cell Biol. 2000; 2(2):76-83. [PMID: 10655586]
- Varlet I, Collignon J, and Robertson EJ. nodal expression in the primitive endoderm is required for specification of the anterior axis during mouse gastrulation. Development 1997; 124(5):1033-44. [PMID: 9056778]
- Lee K, Chen QK, Lui C, Cichon MA, Radisky DC, and Nelson CM. Matrix compliance regulates Rac1b localization, NADPH oxidase assembly, and epithelial-mesenchymal transition. Mol. Biol. Cell 2012; 23(20):4097-108. [PMID: 22918955]
- Radisky DC, Levy DD, Littlepage LE, Liu H, Nelson CM, Fata JE, Leake D, Godden EL, Albertson DG, Nieto MA, Werb Z, and Bissell MJ. Rac1b and reactive oxygen species mediate MMP-3-induced EMT and genomic instability. Nature 2005; 436(7047):123-7. [PMID: 16001073]
- Engler A, Bacakova L, Newman C, Hategan A, Griffin M, and Discher D. Substrate compliance versus ligand density in cell on gel responses. Biophys. J. 2004; 86(1 Pt 1):617-28. [PMID: 14695306]
- Gomez EW, Chen QK, Gjorevski N, and Nelson CM. Tissue geometry patterns epithelial-mesenchymal transition via intercellular mechanotransduction. J. Cell. Biochem. 2010; 110(1):44-51. [PMID: 20336666]
- Miralles F, Posern G, Zaromytidou A, and Treisman R. Actin dynamics control SRF activity by regulation of its coactivator MAL. Cell 2003; 113(3):329-42. [PMID: 12732141]
- Posern G, and Treisman R. Actin’ together: serum response factor, its cofactors and the link to signal transduction. Trends Cell Biol. 2006; 16(11):588-96. [PMID: 17035020]
- Morita T, Mayanagi T, and Sobue K. Dual roles of myocardin-related transcription factors in epithelial mesenchymal transition via slug induction and actin remodeling. J. Cell Biol. 2007; 179(5):1027-42. [PMID: 18056415]
- Zhou J, Wang J, Zhang N, Zhang Y, and Li Q. Identification of biomechanical force as a novel inducer of epithelial-mesenchymal transition features in mechanical stretched skin. Am J Transl Res 2015; 7(11):2187-98. [PMID: 26807167]
- Huber MA, Azoitei N, Baumann B, Grünert S, Sommer A, Pehamberger H, Kraut N, Beug H, and Wirth T. NF-kappaB is essential for epithelial-mesenchymal transition and metastasis in a model of breast cancer progression. J. Clin. Invest. 2004; 114(4):569-81. [PMID: 15314694]
- Chua HL, Bhat-Nakshatri P, Clare SE, Morimiya A, Badve S, and Nakshatri H. NF-kappaB represses E-cadherin expression and enhances epithelial to mesenchymal transition of mammary epithelial cells: potential involvement of ZEB-1 and ZEB-2. Oncogene 2006; 26(5):711-24. [PMID: 16862183]
- Boyle ST, Kular J, Nobis M, Ruszkiewicz A, Timpson P, and Samuel MS. Acute compressive stress activates RHO/ROCK-mediated cellular processes. Small GTPases 2018;:1-17. [PMID: 29455593]